Optimized Multi-Element Analysis of Environmental Waters
App Note / Case Study
Published: June 25, 2024
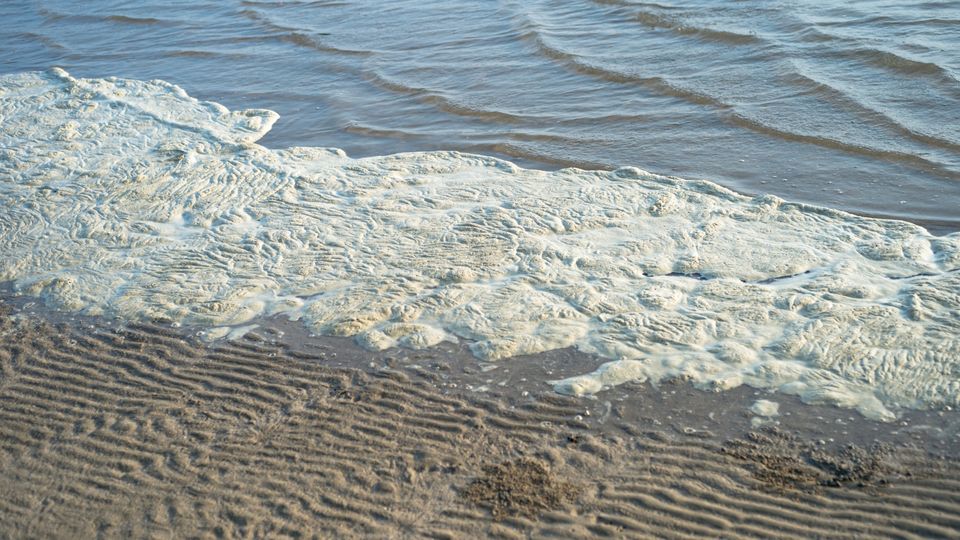
Credit: iStock
The release of toxic substances, such as heavy metals, from domestic and industrial activities poses significant health risks due to their toxicity, persistence and bioaccumulative properties.
Inductively coupled plasma mass spectrometry (ICP-MS) is the preferred technique for analyzing low concentrations of metals in environmental samples. However, high levels of dissolved solids in complex samples can significantly affect the instrument's sensitivity.
This application note describes an optimized method for analyzing a wide variety of water samples using argon gas dilution (AGD) to mitigate matrix effects and streamline sample preparation.
Download this application note to discover:
- How triple quadrupole ICP-MS can enhance the accuracy and sensitivity of heavy metal detection
- The benefits of using AGD for direct analysis of trace elements in complex environmental samples
- How to optimize multi-element determination in surface and sea waters
APPLICATION NOTE 44495
Robust and sensitive multi-element
determination in surface and sea waters using
triple quadrupole ICP-MS
Authors: Tomoko Vincent1
, Naoki Iwayama2
and Tokutaka Ikemoto3
1
Thermo Fisher Scientific, Germany,
2
Seikan Kensa Center Inc., Japan,
3
Thermo Fisher Scientific, Japan
Keywords: Argon gas dilution (AGD),
drinking water, environmental analysis, lake
water, river water, sea water, sensitivity and
robustness, surface waters, TQ-ICP-MS
Goal
To demonstrate the applicability of the Thermo Scientific™
iCAP™ TQe ICP-MS in combination with argon gas dilution
(AGD) for direct analysis of trace elements in complex
environmental samples (i.e., sea water, brackish water,
lake water, or river water).
Introduction
The release of toxic substances into the environment
as a result of domestic or industrial activities is a major
cause of concern, with potential health risks associated
with the toxicity of the chemicals discharged.1
Amongst
environmental pollutants, heavy metals are of interest due
to their known toxicity combined with their persistence and
bio-accumulative properties.2
Although heavy metals can
originate from natural sources, anthropogenic activities,
such as mining, industrial production, and agricultural
activities,3
are typically the main routes of release of these
elements into the environment. To protect human health and
the environment, strict regulatory measures and guidelines
have been established globally and/or specifically for certain
countries, with clear maximum allowable concentrations of
toxic elements in environmental samples (such as sea water
or potable water). As an example, the applicable maximum
contaminant levels established by the Ministry of the
Environment in Japan for potable, sea, and river waters are
summarized in Table 1.
Table 1. Maximum allowable limits (µg·L-1) of inorganic elements in
potable water and environment water in Japan. Numbers annotated
with * apply to river water only.
Table 2. Instrument configuration and operating parameters
Element Potable water Sea and river water
Cd 3 3
Se 10 10
Pb 10 10
As 10 10
Cr (Cr6+) 20 50
B 1,000 1,000*
Zn 1,000 -
Al 200 -
Fe 300 -
Cu 1,000 -
Na 200,000 -
Mn 50 -
Ca, Mg etc.
(Hardness) 300,000 -
Hg 0.5 0.5
In general, Inductively Coupled Plasma Mass Spectrometry
(ICP-MS) is the preferred analytical technique for the
analysis of metals and related contaminants at low levels.
However, the analysis of samples containing higher levels
of dissolved solids (typically above 0.5%) is a known
challenge in ICP-MS. The complexity of the sample matrix
can significantly affect the sensitivity of the instrument,
cause intensity fluctuation of the internal standard
(suppression and drift), and lead to increased system
maintenance with unwanted downtime due to clogging of
the interface cone orifices, torch injectors, or nebulizer tips.
To analyze complex samples, such as sea water,
dilution must be performed, either using liquid dilution
or, alternatively, dilution of the sample aerosol with an
inert gas such as argon. Dilution with argon gas is often
perceived as beneficial because samples can be placed
directly on the autosampler, without the need of a manual,
time-consuming dilution step prior to analysis. However,
reducing the impact of the matrix by sample dilution is not
the only challenge for this analysis. Elements with high first
ionization potentials, such as zinc, arsenic, selenium, and
cadmium, typically suffer from reduced recovery under the
dry plasma conditions observed when using gas dilution.
For all elements, the potential occurrence of interferences
(predominantly polyatomic species) may lead to false
positive results if these are not removed.
This application note describes an optimized analytical
method for the analysis of a variety of water samples
(sea, lake, river, and potable waters) using the iCAP TQe
ICP-MS. To overcome the analytical challenges described
above, this instrument was operated using argon gas
dilution (AGD) and was equipped with the novel PLUS
torch4
. The PLUS torch is a replacement for the standard
torch of the system made from high purity ceramic material.
Experimental
Instrumentation
An iCAP TQe ICP-MS system equipped with a standard
sample introduction system configuration was used
for all analyses. To automate the sample introduction,
a Teledyne™ CETAC™ ASX-560 autosampler (Teledyne
CETAC Technologies, Omaha, NE, USA) was selected. To
remove all potentially occurring interferences, the ICP-MS
was operated in SQ KED and TQ-O2
modes, using the
parameters presented in Table 2.
2
Parameter Value
Nebulizer
Borosilicate glass Micromist (Glass
Expansion, Australia), 400 µL·min-1,
pumped at 30 rpm
Pump tubing Orange – green, 0.38 mm i.d.
Spray chamber Quartz cyclonic, cooled at 2.7 ˚C
Torch PLUS torch
Injector 2.5 mm i.d., quartz
Interface Nickel sampler and nickel skimmer cone with
High Matrix insert
Plasma power 1,550 W
Nebulizer gas 0.30 L·min-1
Additional argon gas 0.68 L·min-1
QCell setting TQ-O2 SQ KED
Gas flow 100% O2
,
0.34 mL·min-1
100% He,
5.5 mL·min-1
CR bias -6.3 V -21 V
Q3 bias -12 V -18 V
Scan settings 0.1 s dwell time, 5 sweeps, 3 main runs
Lens setting Autotuned
Sample uptake 60 s
Wash time 50 s
Total analysis time 3 min
The instrument was equipped with the option to use
Argon Gas Dilution (AGD) to facilitate the analysis of all
types of water samples [including sea water samples with
high total dissolved solids (TDS)] without any liquid dilution
prior to analysis in a single batch.5
To accomplish this, the
gas flow to the nebulizer is reduced, while an additional
flow of argon gas is added to the gas port located at
the spray chamber elbow, resulting in a dilution factor of
~10 times applied to the samples (as derived from the
difference in intensity observed with AGD compared to the
standard tuning of the instrument). Consequently, using
AGD, less sample is introduced to the plasma, and the
plasma is significantly drier (a reduction of the oxide ratio is
typically observed). Overall, the use of AGD leads to more
effective decomposition of the sample constituents and
hence improved matrix tolerance.
The analysis of samples containing high amounts
of TDS can lead to an increased maintenance cycle,
predominantly for the interface cones but also for the
torch. The deposition of salt residues over time can lead
to devitrification, shortening the lifetime of conventional
quartz torches.
Data acquisition and data processing
The Thermo Scientific™ Qtegra™ Intelligent Scientific Data
Solution™ (ISDS) Software was used to create LabBooks
for sample analysis, data acquisition, processing, and
reporting. The Reaction Finder method development tool
automates the selection of optimized collision/reaction gas
selection and product ion mass (if applicable) to ensure
generation of the best quality data through complete
interference removal with minimal user effort. Based on the
selection of the element to analyze from the periodic table,
Reaction Finder will automatically create a method using
optimized analysis settings, including selection of the best
isotope for analysis, acquisition mode (SQ vs. TQ), reactive
gas, and scan type (mass shift vs. on mass).
Sample preparation
Polypropylene bottles were used for the preparation of all
blanks, calibration standards, and samples. Two certified
reference materials (CRM) were used: NASS-7 [sea
water, National Research Council Canada (NRC-CNRC)]
and SLRS-5 (river water, NRC-CNRC). In addition, three
different water samples were collected in and around
Bremen, Germany (sea water, lake water, and potable
water). All water samples were acidified with 2% v/v
HNO3
(Optima™ grade, Fisher Scientific™) after collection.
In addition, the samples were filtered through a 0.45 μm
membrane to remove particles. A total of 30 elements were
analyzed in all samples. All blanks, calibration standards,
and quality control standards (QC) were prepared using a
mixed acid diluent [2% v/v HNO3
and 1% v/v HCl (Optima
grade, Fisher Scientific)] and single element standards
(SPEX™ CertiPrep, Metuchen, NJ, USA), listed in Table 3.
Table 3. Summary of the concentration details of the CCV and CCB QC solutions
Concentration range
in calibration solutions
(μg·L-1)
CCB CCV (μg·L-1) Sea water spike level
(μg·L-1)
Li, Be, Al 2–200 2% HNO3
and 1% HCl 10 20
Ti, V, Cr, Mn, Fe, Co, Ni,
Cu, Zn, As, Se, Mo, Ag,
Cd, Sn, Sb, Ba, Hg, Tl,
Pb, U
1–50 2% HNO3
and 1% HCl 10 20
B, Si 50–2,000 2% HNO3
and 1% HCl 200 200
Mg, K, Ca 10,000–200,000 2% HNO3
and 1% HCl 40,000 100,000
Sr 2–200 2% HNO3
and 1% HCl 10 1,000
3
An internal standard solution, containing 100 μg·L-1 Y, In,
and Tb in 2% v/v HNO3
and 5% v/v isopropanol (IPA) was
added to all samples prior to entry to the spray chamber,
via a T-piece with a mixing ratio between the internal
standard and the samples of 1:1. The addition of IPA to the
plasma enhances the sensitivity of some elements, such
as arsenic and selenium, resulting to improved results, as
reported previously.6
Details of the measurement modes, acquisition
parameters, and internal standards used for each element
are summarized in Table 4.
For ongoing quality control, Continuing Calibration
Verification (CCV) and Continuing Calibration Blank (CCB)
solutions were periodically analyzed throughout the entire
measurement sequence.
Results and discussion
Sensitivity and linearity
Table 4 summarizes the Instrument Detection Limits (IDLs)
obtained, together with the coefficient of determination
(R2
) for all the elements analyzed in this study. The IDLs
were calculated as three times the standard deviation of
ten replicate measurements of the calibration blank. As no
manual dilution was performed prior analysis, these IDLs
directly correspond to the method detection limits (MDL).
The MDLs obtained were significantly below the maximum
limitation of the Japanese Ministry of the Environment
regulations (Table 1).
Accuracy, precision, and robustness
Interference removal
In general, determination of the heavy metals of greatest
concern (e.g., cadmium, tin, mercury, lead) requires
analysis at µg∙L-1 levels or lower. At the same time, typical
water samples contain high amounts of Na, K, Ca, and Mg
(often in the region of several hundred mg∙L-1). These major
elements, together with their corresponding anions, can
create intense and complicated interferences on the low
concentration analytes. Moreover, unexpected elements,
such as the lanthanides, can often cause positive bias
on the results for key elements (for example, 150Nd2+
interference on 75As) even at relatively low concentrations.
Therefore, it is important to ensure complete interference
removal to avoid false positive results by using triple
quadrupole ICP-MS where appropriate.
Table 4. Summary of analysis modes, calibration results, and MDLs
for all target analytes. The concentration range for R2
was shown in
Table 3.
Analyte Mode Internal
standard
Coefficient of
determination
(R2)
MDLs
(μg·L-1)
7Li KED 115In 0.9994 0.340
9Be KED 115In 0.9999 0.471
11B KED 115In 0.9992 0.573
24Mg KED 115In 0.9999 3.000
27Al KED 115In 0.9997 0.579
28Si as 28Si16O
at m/z 44 TQ-O2
89Y 0.9991 5.200
39K KED 115In 0.9998 57.174
44Ca KED 115In 0.9995 25.043
49Ti as 49Ti16O
at m/z 65 TQ-O2
89Y >0.9999 0.043
51V as 51V16O
at m/z 67 TQ-O2
89Y >0.9999 0.034
52Cr KED 115In >0.9999 0.162
55Mn KED 115In >0.9999 0.016
56Fe KED 115In 0.9996 0.372
59Co KED 115In >0.9999 0.015
60Ni KED 115In 0.9965 0.067
63Cu KED 115In >0.9999 0.048
66Zn KED 115In >0.9999 0.105
75As as 75As16O
at m/z 91 TQ-O2
115In >0.9999 0.018
80Se as 80Se16O
at m/z 96 TQ-O2
115In >0.9999 0.029
88Sr as 88Sr16O
at m/z 104 TQ-O2
115In >0.9999 0.053
98Mo as 98Mo16O
at m/z 114 TQ-O2
115In >0.9999 0.016
107Ag KED 115In >0.9999 0.011
111Cd as 111Cd
at m/z 111 TQ-O2
159Tb >0.9999 0.005
118Sn KED 159Tb >0.9999 0.067
121Sb KED 159Tb >0.9999 0.047
138Ba KED 159Tb >0.9999 0.038
202Hg KED 159Tb 0.9999 0.010
205Tl KED 159Tb >0.9999 0.001
208Pb KED 159Tb >0.9999 0.004
238U KED 159Tb >0.9999 0.003
4
As an example, the analysis of titanium is illustrated
in Figure 1 and shows how TQ-ICP-MS can help to
overcome potential interferences in calcium rich sample
matrices. In this case, the most abundant isotope of
titanium (48Ti) is affected by isobaric overlap with 48Ca.
Although the use of ammonia is widely recognized as an
effective way to remove this potential bias, this reactive
gas is not commonly found in laboratories performing
analytical testing of water samples due to health and
safety restrictions. However, the use of O2 as the reaction
gas with a different titanium isotope (49Ti) provides an
effective alternative approach (48Ti cannot be used with
an O2 cell gas due to reaction between 48Ca+ and O2
).
With Q1 set to transmit 49Ti+ and Q3 set to transmit the
49Ti16O+ product ion, interference from 48Ca is completely
avoided and any 48Ca1
H+ interference on 49Ti is filtered
out in the collision / reaction cell (Q2). As a result of
mass filtration in the first quadrupole, 65Cu+, which would
otherwise be a potential interference on the 49Ti16O+
product ion, is eliminated before the ion beam enters
Q2. Table 5 compares the results between He KED
mode and TQ-O2 mode in sea water and drinking water,
respectively. As the sea water analyzed in this study
contained >400 mg·L-1 of Ca, full interference removal
for 49Ti could not be accomplished using He KED
mode alone. The results in this mode were >140 times
higher than those obtained in TQ-O2 mode. This false
positive result mainly originates from unresolved 48Ca1
H+
interference, with potential additional interference from
35Cl14N+. In contrast, TQ-O2 mode allowed significantly
lower backgrounds to be obtained and more accurate
results compared to He KED mode where false positive
results were observed. Similar results were obtained for
drinking water, which although containing a lower amount
of calcium, was still positively biased in He KED mode.
Figure 1. Schematic diagram showing the use of TQ-O2
mode to
selectively analyze titanium in the presence of calcium induced
interferences
Table 5. Comparison of results obtained with He KED and TQ-O2
modes using AGD when analyzing titanium (49Ti) in samples
containing high amounts of calcium
Ca amount
(μg·L-1)
Ti concentration results (μg·L-1)
He KED
at m/z 49
TQ-O2
at m/z 65
as 49Ti16O
Sea water >400,000 130 0.9
Drinking water >40,000 3 <MDL
As part of this study, two certified reference materials
for sea water (NASS-7) and river water (SLRS-5), as well
as various water samples, were analyzed to assess the
method performance. In total, 171 samples were analyzed
(the details are shown in Table 7). The results for both
CRMs were in excellent agreement with the reference
values (Table 6), except for chromium and iron, for which
the concentrations found in the CRM value were close to
or below the MDL levels. To ensure accurate and reliable
analysis, samples were spiked and the recoveries were
determined. (The spike concentrations are shown in
Table 3.)
Set to product ion
mass (m/z 65)
Filled with
reactive gas (O2
)
Set to analyte
mass (m/z 49)
65[
49Ti16O]+
48Ca1
H+
65Cu+
49Ti+ 65[
49Ti16O]+
49Ti+,
48Ca1
H+
Q1
Q2
Q3
5
Table 6. Quantification of multiple elements in several water samples. All data annotated with * are known reference values (expected values) and **
are indicative (non-certified) values.
Analyte
NASS-7 sea water (µg·L-1) SLRS-5 river water (µg·L-1) Unknown samples (µg·L-1)
CRM value Measured
(n=5)
Spike
recovery (%) CRM value Measured
(n=15) Lake water Potable water
7Li - 146 ± 12.8 108 - 0.4 ± 0.05 3.0 6.2
9Be - <MDL 84 0.005* <MDL <MDL <MDL
11B 3,750 ± 120 3856 ± 105(a) 94 - 22.8 ± 3.9 137.5 20
24Mg - 1,142,000 ± 44(a) 100 2,540 ± 160 2,570 ± 140 6,410 4,160
27Al - 1.2 ± 0.15 94 49.5 ± 5.0 45.8 ± 1.52 19.8 1.04
28Si as 28Si16O
at m/z 44 - 171.2 ± 47.6 111 - 1,959 ± 40(a) 717.9 9,740.02(a)
39K - 347,400 ± 9.3(a) 82 839 ± 36 887 ± 10 10,320 2,540
44Ca - 397,400 ± 6.4(a) 106 10,500 ± 400 10,935 ± 347 50,500 42,650
49Ti as 49Ti16O
at m/z 65 - 0.9 ± 0.07 101 - 0.3 ± 0.07 0.4 <MDL
51V as 51V16O
at m/z 67 1.27 ± 0.08** 1.37 ± 0.12 101 0.317 ± 0.033 0.327 ± 0.0.04 0.1 0.07
52Cr 0.107 ± 0.016** <MDL 95 0.208 ± 0.023 0.230 ± 0.032 <MDL <MDL
55Mn 0.75 ± 0.06 0.8 ± 0.15 90 4.33 ± 0.18 4.22 ± 0.21 89.77 0.06
56Fe 0.351 ± 0.026 <MDL 103 91.2 ± 5.8 93.1 ± 2.9(a) 23.66 2.01
59Co 0.0146 ± 0.0014 <MDL 109 0.05* 0.052 ± 0.003 0.05 0.03
60Ni 0.248 ± 0.018 0.25 ± 0.084 101 0.476 ± 0.064 0.389 ± 0.015 0.5 0.5
63Cu 0.195 ± 0.014 0.182 ± 0.046 99 17.4 ± 1.3 17.5 ± 0.92 1.77 12.9
66Zn 0.42 ± 0.08 0.43 ± 0.18 88 0.845 ± 0.095 0.90 ± 0.036 161.7(a) 12.6
75As as 75As16O
at m/z 91 1.26 ± 0.02** 1.24 ± 0.09 96% 0.413 ± 0.039 0.39 ± 0.02 0.71 0.07
80Se as 80Se16O
at m/z 96 - 0.24 ± 0.09 101% - 0.082 ± 0.003 0.05 0.07
88Sr as 88Sr16O
at m/z 104 - 6,436 ± 233(a) 102% 53.6 ± 1.3 51.9 ± 0.86 216.5 120.37
98Mo as 98Mo16O
at m/z 114 9.29 ± 0.4 9.49 ± 0.54 107% 0.5* 0.52 ± 0.03 0.1 0.06
107Ag - 0.002 ± 0.004 88% - <MDL <MDL <MDL
111Cd as 111Cd
at m/z 111 0.0161 ± 0.0016 0.0153 ± 0.002 102% 0.0060 ± 0.0014 0.007 ± 0.001 0.01 0.01
118Sn - <MDL 84% - <MDL <MDL <MDL
121Sb - 0.227 ± 0.009 96% 0.3* 0.33 ± 0.01 0.09 0.01
138Ba - 5.58 ± 0.055 104% 14.0 ± 0.5 15.0 ± 0.18 232.71(a) 16.81
202Hg - 0.034 ± 0.047 101% - 0.03 ± 0.02 0.03 0.03
205Tl - 0.011 ± 0.008 103% 0.003 ± 0.002 0.01 <MDL
208Pb 0.0026 ± 0.0008 0.0025 ± 0.0014 98% 0.081 ± 0.006 0.09 ± 0.002 0.04 0.11
238U 2.92 ± 0.42 3.2 ± 0.264 106% 0.1* 0.097 ± 0.004 0.07 0.01
Results annotated with (a) are higher than the maximum calibration standard concentration, but nonetheless show good spike recovery results because of the wide linear
range of the detector.
6
Table 7. Analysis procedure for a total of 171 samples over 9 hours
Batch
component Sample detail Total number of
samples
1 Standard calibration solution
and ICV 14
2 Sea water and spiked sea water 20
3–8
5 samples each of lake water,
river water, drinking water and
3 sea water samples
100
N/A QC checks (CCB, CCV for
different groups of elements) 37
Robustness
For reliable analysis in high-throughput analytical testing
laboratories, it is important that the results obtained
are accurate and precise throughout long analytical
run sequences consisting of different sample types.
Commonly, quality control (QC) standards containing
known concentrations of all the analytes of interest are
analyzed periodically during each analysis batch to monitor
method performance.
Table 7 shows the analysis procedure for this study. To
simulate a high sample volume, a larger sample batch was
scheduled for analysis containing all the water samples
previously analyzed. The batch contained several blocks
of unknown samples and required QC checks, as shown in
Table 7. The total number of solutions analyzed was
171 samples (including 120 unknown samples), requiring a
total analysis time of approximately 9 hours. The batch was
repeated on 12 consecutive working days.
The relative standard deviation of all CCVs (n = 5 per batch)
showed excellent recovery (within 92% to 104%) with a
relative standard deviation of ±3.5% within the batch. The
response of the internal standards in one selected analysis is
shown in Figure 2. All internal standards showed reliable and
predictable recovery (within approximately 69% to 122%)
over the entire runtime of the batch, demonstrating robust
analytical performance. It is important to note that those
samples causing a suppression of the internal standard
were, in every case, undiluted sea water. Whereas an internal
standard recovery of around 70% still allows for accurate
correction of matrix effects and potential drift, it is far more
important that the signal of the internal standard immediately
and reliably returns to expected levels (approximately
110% in this example) when switching back to lower matrix
samples (e.g., 2% HNO3
for QC checks or less saline water
types). This ultimately demonstrates the system´s ability to
effectively analyze a variety of different sample types with
vastly different TDS load in a single batch.
The analysis of samples such as undiluted sea water also
has a significant impact on all components of the sample
introduction system. While clogging of the cones through
deposition of material is greatly reduced by using AGD
other components of the system (i.e., the torch and injector)
are still in direct contact with the sample. Prolonged
analysis of such sample matrices is known to cause
devitrification, which ultimately leads to more frequent
replacement/exchange of the torch.
Figure 2. Response of the internal standards in a batch covering about ~9 hours of uninterrupted analysis of 171 samples. Samples with lower
recovery (approx. 70%) correspond to undiluted sea water.
7
Find out more at thermofisher.com/TQ-ICP-MS
For Research Use Only. Not for use in diagnostic procedures. © 2021 Thermo Fisher Scientific Inc. All rights reserved. SPEX
is a trademark of CertiPrep. Teledyne CETAC is a trademark of Teledyne Cetac Technologies. All other trademarks are the property of
Thermo Fisher Scientific and its subsidiaries. This information is presented as an example of the capabilities of Thermo Fisher Scientific
products. It is not intended to encourage use of these products in any manners that might infringe the intellectual property rights of
others. Specifications, terms and pricing are subject to change. Not all products are available in all countries. Please consult your local
sales representatives for details. AN44495-EN 0621C
In this study, the iCAP TQe ICP-MS was equipped with the
novel PLUS torch, a direct replacement of the standard
quartz torch, with identical geometry, made from a high
performance ceramic material. This material is frequently
used for torches used in ICP-OES and has been proven in
the field to last significantly longer when routinely analyzing
challenging sample matrices. The use of the PLUS torch also
provides a number of additional advantages for analysis using
ICP-MS. Due to the opaque color of the material, the plasma
temperature is slightly increased, which enables more effective
decomposition of the sample matrix. In conjunction with
sample dilution using AGD, greater robustness is achieved,
which is reflected in improved long term stability of the internal
standard signal, as illustrated in Figure 2.
Figure 3 shows an image of the PLUS torch and injector tip
after repeating the aforementioned batch analysis over 12
consecutive days. As shown, the torch body and injector are
both in perfect condition, with no visible deposition of material.
The use of the PLUS torch for such analysis facilitates more
consistent system performance with reduced maintenance
intervals and less instrument down time, ultimately enabling
higher productivity and reduced cost per sample.
Figure 3. PLUS torch (left) and tip of the injector (right) after the
repeated analysis of a mixed batch containing all sample types over
12 consecutive days
Conclusions
The iCAP TQe ICP-MS, equipped with the PLUS torch
and operated using AGD, was employed to analyze
30 elements in different environmental water samples,
including sea water, without any upfront manual or
automated dilution. A suite of major and toxic elements
commonly analyzed in water were determined in these
samples. The analytical method was rigorously tested
for performance, and the results obtained clearly
demonstrated the following analytical advantages:
• The iCAP TQe ICP-MS allowed the aspiration of all
sample matrices without prior manual dilution and
allowed excellent MDLs to be obtained, eliminating the
need for labor-intensive manual sample dilution and
accelerating the sample throughput.
• Excellent agreement with representative CRMs was
achieved. Additionally, spike recovery tests revealed
recoveries between 84 to 110%, in line with the expected
values in most regulatory methods.
• The TQ-O2
operating mode allowed for selective and
precise determination of multiple elements, including Si,
Ti, V, As, Se, Mo, and Cd, in all the samples analyzed.
• Excellent CCV recovery results (92 to 104%) and stable
and consistent internal standard response were obtained
across a sample batch containing 171 of samples,
demonstrating the reliability of the approach.
• The novel PLUS torch allowed consistent analysis of a
large number of samples, including high TDS samples
such as sea water, over twelve days without any loss of
performance or need for maintenance.
• In summary, the iCAP-TQe ICP-MS, operated in TQ-O2
and SQ KED modes and equipped with the AGD option,
represents a viable analytical configuration for analytical
testing laboratories where a variety of environmental
samples, in high numbers, are required to be analyzed
for their elemental content and where instrument uptime
and reliable, consistent analytical results are vital.
References
1. Zhou, J. et al. Mine waste acidic potential and distribution of antimony and arsenic in
waters of the Xikuangshan mine, China. Applied Geochemistry, 2017, 77, 52-61.
2. Cabral Pinto, M. et al. Human Health Risk Assessment Due to Agricultural Activities
and Crop Consumption in the Surroundings of an Industrial Area. Exposure and Health,
2020, 12, 629-640.
3. Birka, M. et al. Tracing gadolinium-based contrast agents from surface water to drinking
water by means of speciation analysis. Journal of Chromatography A, 2016, 1440,
105–111.
4. Thermo Fisher Scientific. Product Spotlight 44485: Thermo Scientific iCAP Qnova
Series ICP-MS PLUS Torch for Improved ICP-MS Analysis of Challenging Samples.
5. Thermo Fisher Scientific. Technical Note 43202: Analysis of High Matrix Samples using
Argon Gas Dilution with the Thermo Scientific iCAP Q ICP-MS.
6. Thermo Fisher Scientific. Application Note 43250: Dramatically Improved Performance
of Arsenic and Selenium Determination using the Thermo Scientific iCAP Q ICP-MS with
Methane Addition.
Brought to you by
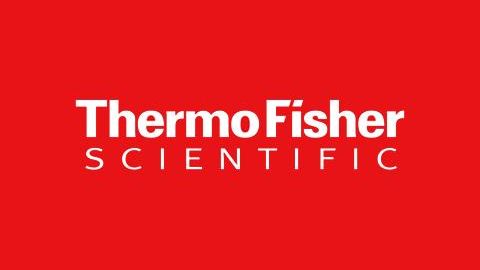
Download this App Note for FREE Below!
Information you provide will be shared with the sponsors for this content.
Technology Networks or its sponsors may contact you to offer you content or products based on your interest in this topic. You may opt-out at any time.
Experiencing issues viewing the form? Click here to access an alternate version